High Voltage Capacitors Designed To Avoid Catastrophic Failure Modes Capacitorss
User Manual: capacitorss-avoid-catastrophic-failure Maxwell
Open the PDF directly: View PDF .
Page Count: 6
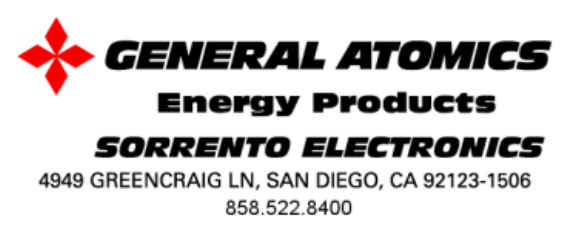
GENERAL ATOMICS ENERGY PRODUCTS
Engineering Bulletin
High Voltage Capacitors
Designed to Avoid
Catastrophic Failure Modes
F. W. MacDougall G. L. McKee, J.B. Ennis, R.A. Cooper
Maxwell Energy Products, Inc. San Diego, CA.
R. M. Ness, - Cymer, Inc. San Diego CA.
W. A. Reass – Los Alamos National Laboratories
Presented at:
IEEE Pulsed Power Conference
June 1999
© 1999 IEEE
www.gaep.com
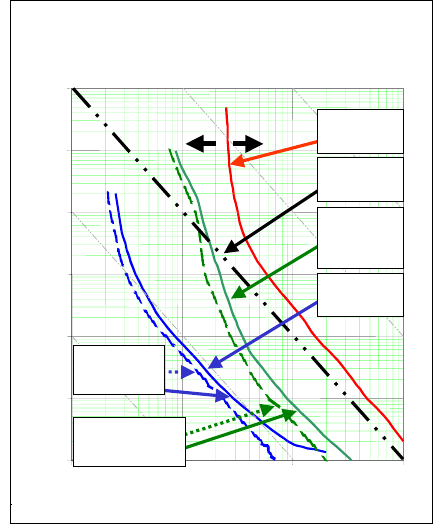
High Voltage Capacitors Designed to
Avoid Catastrophic Failure Modes
F. W. MacDougall G. L. McKee, J.B. Ennis, R.A. Cooper
Maxwell Energy Products, Inc. San Diego, CA.
R. M. Ness, - Cymer, Inc. San Diego CA.
W. A. Reass – Los Alamos National Laboratories
Abstract:
A major concern in the operation of high voltage
capacitors is the failure mode at end of life. While
progress has been made in this area at lower voltages,
little has changed for high current capacitors operating
above 30 kV in steady state and pulsed power
applications. Based on a new development at Maxwell
Energy Products, Inc., it is now possible to build
capacitors that can operate with a high degree of safety at
high voltages. The capacitors reach the normal end of life,
while avoiding the catastrophic failures, collateral
damage, and unscheduled maintenance, associated with
the normal failure mode of today’s high voltage
capacitors. This paper discusses the problem, capacitor
failure modes, capacitor designs and describes a solution
to the problem of catastrophic high voltage capacitor
failures.
I. HISTORY
Over the years, the emphasis on pulsed power
capacitor development has been on cost. The minimum
requirements are normally expressed in terms of life
expectancy, inductance, and in some cases volume.
Some specifications go as far as to require that testing be
done to demonstrate the quoted capability while other
specifications have relied on extrapolated data from the
manufacturer as an evaluation method.
Few specifications have reference to the failure mode
associated with at capacitor. Probably the primary reason
this occurs is that no one wants to dwell on the inevitable
demise of the equipment. Until recently, it has been
difficult to get a manufacturer to accept a contract where
the capacitor failure mode is specified.
II. POWER FACTOR CAPACITORS
In the 1970s there was a major change in high voltage
power factor correction (PFC) capacitors that resulted in
an intensive investigation of the capacitor time current
characteristics [1,2]. The dielectric fluid in the capacitors
was changed from non-flammable PCBs to flammable
fluids like MIPB.
The time current characteristics for a high voltage
PFC capacitors as well as typical expulsion and current-
limiting fuses [3] is shown in Figure 1. There are three
general areas of the curves shown. If the curves are
extended out long enough in time, the devices will reach
“thermal stability” and the curves become constant
current curves. At the fast end of the curve, the curves
approach a constant I2t. The I2t value is often referred to
as the “Action”. The constant Action section of the curve
results from the inability of the devices to dissipate energy
from the faulted area in the allotted time frame.
Connecting the constant current and constant Action
sections is the middle of the time current characteristic
(TCC) curve.
0.01
0.1
1
10
100
1000
10000
10 100 1000 10000
Log Time in Seconds
High Voltage (15kV)
Power Factor Correction Capacitor & Fuse
Time Current Characteristics (TCC)
Log Current in Amperes
Safe Unsafe
10Amp K Fuse
Min. Melt
Max Clear
35Amp NXC Fuse
Min. Melt
Max Clear
1,000,000
Amp2 Sec
Capacitor
TCC
Current
Limiting
Fuse
Expulsion
Fuse
Figure 1. TCC CURVES
Early on, it was realized that fuses could be used to
minimize the collateral damage done when a capacitor
fails. For fused in general, there is a point where they
will start to melt and a point at which they will always
clear. If the fuse will clear before the case will rupture,
the capacitor is properly protected. Note that the
maximum clear curve for the expulsion fuse (10 amp K
fuse) shown in Figure 1 approaches a minimum time to
clear. This type of fuse will wait for a current zero to
clear the circuit which can be as long as 0.8 cycles or
0.013 seconds at 60 Hz.
There is second limit that must be considered when
fusing a PFC capacitors. If there is more than 9300
kVAR or 25 kJ in parallel, current limiting fuses must be
used since the expulsion fuse will not interrupt the parallel
discharge of energy into the capacitor that has failed and
the energy level will be high enough to rupture the
capacitor case due to stored energy in the bank.
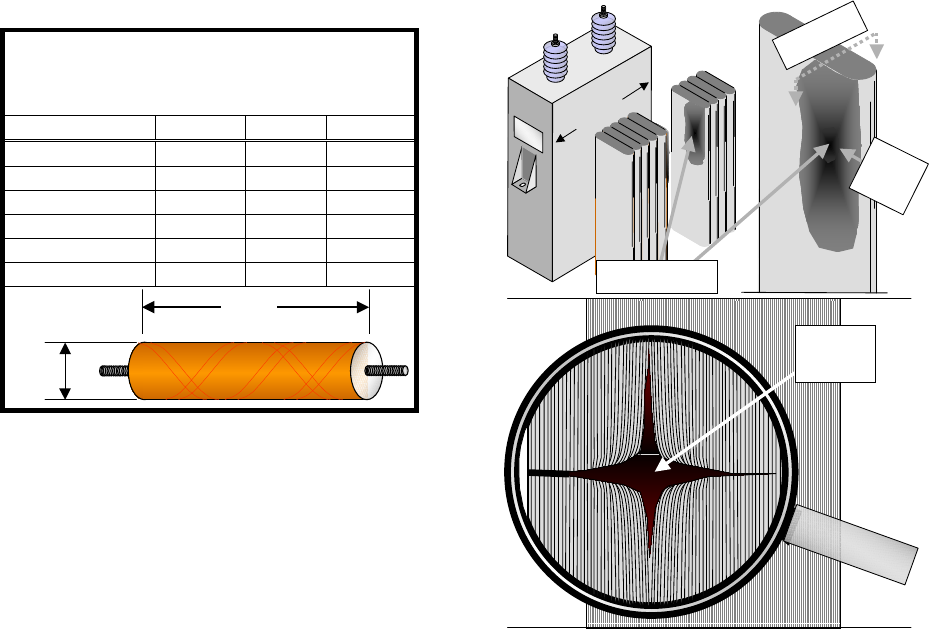
III. PULSE POWER CAPACITORS
Unlike PFC capacitors, pulse power capacitors come
in a wide variety of designs for very specific applications.
No group has been studied as extensively as PFC
capacitors, with the possible exception of the capacitors
developed for NIF, but the principles developed during
the PFC capacitor study are applicable to energy storage
capacitors.
Fuses are available for pulsed power capacitors [4].
The time frame of interest for single shot pulse power
fuse is short and falls into the constant Action area.
Typical values are shown in Figure 2.
Maxwell
Value Range for Standard
22kV Capacitor Fuses
Item Small Large Units
Rated Current 25 59 Amps
Inductance 250 150
nH
20°C Res. 74 13 mOhm
250°C Res. 145 25 mOhm
Action (Min) 1.5k 54k Amp2 Sec
Action to Clear 6.3k 222k Amp2 Sec
2 3/8”D 12”
Figure 2. PULSE POWER CURRENT LIMITING
FUSES
Considering the Action integral in terms of Amp2 Sec
as it applies to energy storage capacitors is a little
difficult, but the units of measure can be changed. For the
evaluation of the amount of energy that can be absorbed
by a capacitor the values could be state as:
Amp2 Seconds = Joules/Ω (1)
Where:
Joules ≡ Energy Stored
Ω ≡ The failure resistance
The energy stored in a capacitor or capacitor bank is
relatively easy to calculate. Determining the fault
resistance is not. The resistance of interest is not the fault
circuit resistance but the resistance at the fault point inside
the capacitor that will generate the arc plasma and from
which no heat will escape. While this value is difficult to
measure, it can be said that capacitors with low resistance
faults can absorb more energy than those with high
resistance faults before the case ruptures, because the
energy is dissipated away from the fault point.
Figure 3 shows a typical foil capacitor that has failed
and has been dissected. A typical failure spreads
vertically because that is the way the layers are
mechanically set up and it penetrates horizontally through
layer after layer of dielectric and electrode picking up
energy as it penetrates each layer. As the layers of
electrodes are penetrated, they are also mechanically and
electrically interconnected. The resistance between the
foils after the fault is the resistance of in Formula 1. The
shorting of foils during the failure process is an
inconsistent process with a wide variation in resistance
that changes in response to current surges. However,
experienced capacitor designers can design capacitors that
will fair better than others in the dynamic environment of
an internal fault.
13”
Cut Here
View
Here
Failure Point
Failure
Point
Figure 3. FOIL ELECTRODE CAPACITOR
DISSECTION
Foil electrode pulsed power capacitors are available
today operating between 10 kV and 100 kV, with peak
currents in the ½ M Amp range, and storing 60 kJ that do
not tend to rupture when they fail as terminal to terminal
shorts. This can be attributed to the internal design and
construction of the capacitors.
Self-clearing capacitors utilize metallized electrodes
that are only a few hundred angstroms thick. Should the
dielectric fail, the capacitor is designed so that the fault
current will clear away the electrode before any
significant current can flow through the fault. Metallized
electrode capacitors are not self-clearing under all
conditions of operation. One way to defeat the self-
clearing mechanism is to increasing the voltage across the
capacitor until it shorts.
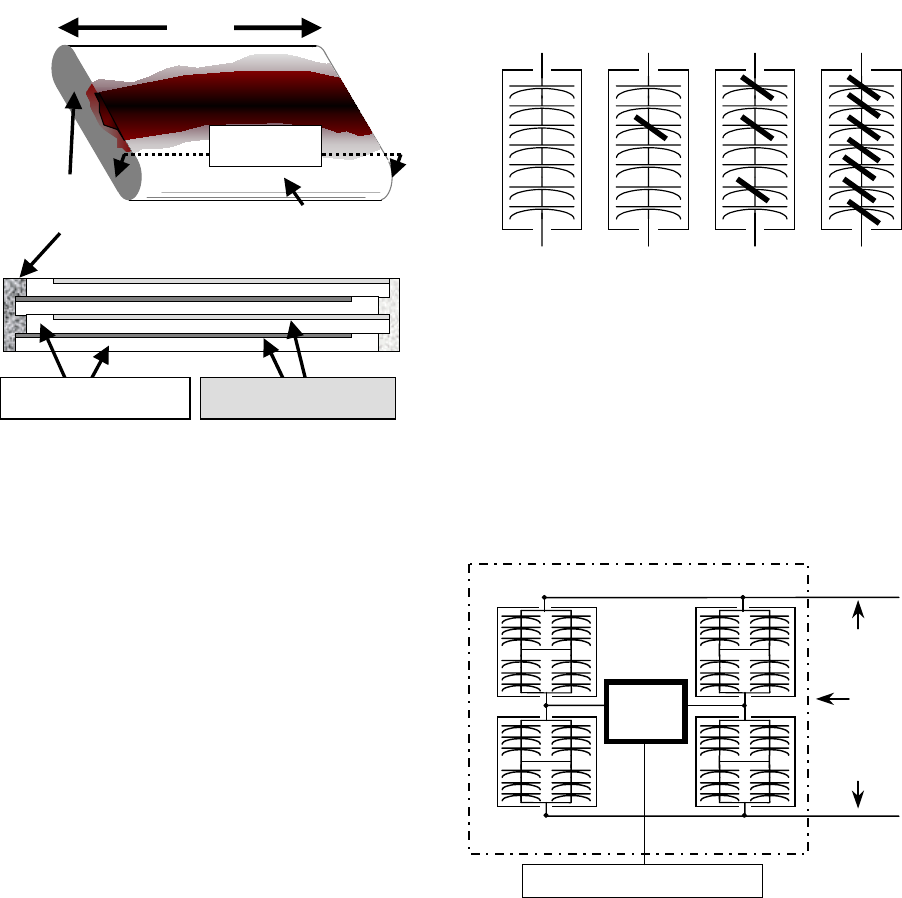
If the self-clearing mechanism is defeated, the fault
area looks similar to that shown in Figure 4. The lower
part of Figure 4 shows the layers inside a typical
metallized electrode capacitor. The black area going
from the endspray at one end of the winding to the other
is typical of what happens when the self-clearing
mechanism is defeated. Since the fault path is several
inches long, the relative resistance of the fault is high.
The amount of energy that the capacitor can absorb with
this type of fault without rupturing is very limited. The
larger metallized electrode energy storage capacitors store
well over 25 kJ and tend to rupture the case if a fault like
that shown in Figure 4 occurs inside the capacitor.
Dielectric ~8µm Electrode ~300Å
~5”
Cut Here
View Here
End
Spray
Figure 4. METALLIZED ELECTRODE
CAPACITOR DISSECTION
Again, experienced capacitor designers have
developed commercially available capacitors for pulsed
power application that do not suffer from this failure
mode. The capacitors are available at voltages of up to
40kV.
IV. HIGH VOLTAGE CAPACITORS FOR
CONTINUOUS PULSED POWER
OPERATION
For some applications, capacitor banks are designed
with high voltage capacitors that must operate under
continuous pulse power duty. Often, the inductance and
resistance of the fuse as listed in Figure 2 will be more
than can be tolerated by the machine design parameters.
Whether or not fuses are used, the failure of a capacitor
comes as a surprise and is often accompanied by an
unscheduled equipment shutdown.
The capacitors used are typically designed for long
life and, as a result operate at fairly low stresses compared
to their high energy density single pulse counterparts. As
shown in Figure 5, the failure normally progresses from a
single shorted internal series section (5b) to the point
where about half the windings are shorted (5c) and at that
time, the remaining windings tend to short
simultaneously. Generally, because of the high peak
currents associated with pulse power applications, the
failed capacitor will not reach thermal equilibrium and the
failure will progress. The time it takes to for the capacitor
to progress from the initial fault (5b) to the point where
the capacitor is a dead short (5d) is significant. During
this time, there may be arcing at the failure point and gas
generated inside the capacitor. If this situation is allowed
too continue for any length of time, it is likely that the
capacitor case will rupture resulting in the unscheduled
shut down of the equipment.
abcd
aNormal Capacitor
bFirst Section Failure
c< Half the sections failed (Not a usual
condition for high energy density
pulsed power capacitors)
dCapacitor shorted terminal to terminal.
Figure 5. TYPICAL FAILURE PROGRESSION IN
A HIGH VOLTAGE FOIL ELECTRODE
PULSED POWER CAPACITOR
C1
C2C4
Unbalance
Threshold
Circuit
Unbalance Detection Output
-
+
Terminal
Case
Terminal
C3
Figure 6. HIGH VOLTAGE CAPACITOR WITH
AN INTERNAL UNBALANCE DETECION CIRCUIT
Figure 6 is a schematic of high voltage capacitor with
an internal unbalance detection scheme. As long as the
impedance of C1/C2 = C3/C4, there is no voltage across

the unbalance threshold circuit. If one of the internal
series sections shorts, the balanced circuit will become
unbalanced and there will be a signal. A typical threshold
detector is shown in Figure 7. Here the unbalance signal
is rectified and accumulated on a capacitor until the
voltage is high enough to cause the neon lamp to flash.
The output of this circuit is a series of light pulses with
the flash rate increasing as the failure in the capacitor
progresses.
Over Voltage Protection
Rectifier Circuit
Signal Accumulator
(Capacitor)
Threshold Detector
(neon lamp)
Unbalance Signal Input
Light Pulse Output
If one of the groups of internal capacitors sections in
Figure 6 starts to go through the failure sequence of
Figure 5, the flash rate can be used to identify the initial
internal capacitor section failure. It can also be used to
determine if the internal failure has progressed beyond the
initial section failure.
For many applications, capacitors can be designed so
that the capacitors will remain stable for some period of
time after the initial fault has taken place. That being the
case, an ALARM function associated with the initial
internal capacitor section failure, can be used to indicate
that the capacitor needs to be change out at the next
scheduled maintenance. Also, if the failure progresses
from the initial internal section failure to the point where
a major fault is of concern, a second signal level can be
used to shut down or STOP the equipment before a
catastrophic failure takes place.
Figure 7. TYPICAL THRESHOLD DETECTOR
(Relaxation Oscillator)
Pulse Count
Comparison Circuit
TD
TD
TD
Normal
Alarm
Stop
Unbalance Control
Capacitor
Capacitor
Voltage Divider
High Voltage Bus
Ground Potential Bus
Pulse
Counter
Optical
Output
Pulse
Counter
Pulse
Counter
Pulse
Counter
Pulse
Counter
Pulse
Counter
Figure 8. SCHEMATIC DIAGRAM OF AN UNBALANCE DETECTION CIRCUIT
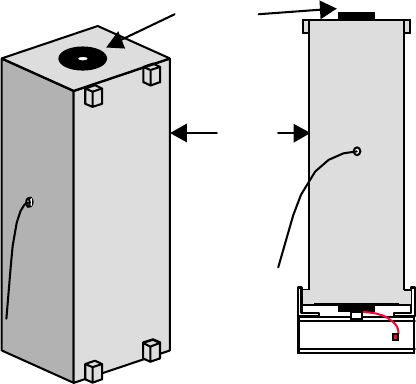
In order to have an ALARM and STOP function, the
capacitor must be designed to carry the available current
after the initial internal failure has occurred. Also, the
circuit must be sensitive to the fault level. The schematic
of Figure 8 shows a typical schematic for a high voltage
capacitor bank using an unbalance detection scheme to
monitor the condition of the capacitors. The capacitors in
the bank each have two internal threshold detectors (TD)
feeding into a single optical output. The light output is
fed into a pulse counter that keeps track of the condition
of the capacitors.
The unbalance signal associated with the failure of an
internal capacitor section can be calculated in terms of the
bus voltage. In pulse power applications, the bus voltage
can vary significantly, operating at different levels,
charging and discharging the capacitor. In the circuit of
Figure 8, a separate capacitive voltage divider is used to
determine what voltage signal is on the bus.
A separate circuit compares the output from the bus
to the output from the capacitors to determine the relative
unbalance each of the capacitors in the bank. The output
of the comparison circuit is a set of contacts that indicate
that the situation is NORMAL, or an ALARM for the
initial failure of an internal capacitor section, or a STOP
signal that will be generated if the internal failure
progresses to the point of concern.
Figure 9 is an example of a high voltage capacitor
might looks like with an internal unbalance detection
scheme and fiber optic output. At the time of this
writing, the unbalance detection scheme with its controls
is still under development. Patent applications have been
filed. The schemes that are being evaluated at this time
are application specific. It is believed that this effort will
result in the development of equipment that can be used in
general applications
V. CONCLUSIONS
An understanding the failure modes of pulse
discharge capacitors can allow users to avoid the
problems associated with capacitor failures. There is
equipment available to day that will allow the user to
avoid most catastrophic failures in a wide variety of
applications. These include the use of fuses, as well as
properly applied foil electrode and metallized electrode
capacitors. One area where there is a lack of good
solutions is the high voltage repetition rate capacitor
banks discharging in microseconds. Here, the unbalance
detection scheme described above is designed to fill the
void and allow continuous operation with minimal
disruptions due to failing capacitors.
Capacitor on
Mounting Stand
Fiber
Optic
Cable
Plastic
Case
Terminal
Figure 9. HIGH VOLTAGE PLASTIC CASE
CAPACITOR WITH AN INTERNAL UNBALANCE
DETECTION SCHEME AND A FIBER OPTIC
OUTPUT
VI. REFERENCES
[1] Lawrence M. Burrage, Shunt Capacitor Rupture
Prevention – Large Bank Applications, IEEE
A76 366-5
[2] Lawrence M. Burrage, Capacitor Tank-Rupture
Prevention – I2t Considerations, IEEE Transactions
on Power Apparatus and Systems, Vol. PAS-97, no 2,
Mar/Apr 1978, IEEE No. F 77 567-1
[3] Cooper Power Systems Reference Data: R230-91-1,
R240-91-2, R240-91-38.
[4] Maxwell Energy Products – High Energy Capacitor
Protection Fuse Selection Guide.